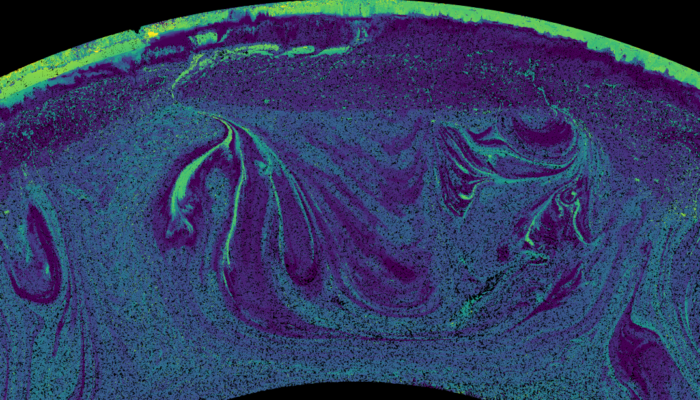
Continents are essential for the development and survival of life on Earth. However, as surprising as it may sound, there did not exist a planetary scale numerical model to show the formation of the oldest continents until the recent study ‘Growing primordial continental crust self-consistently in global mantle convection models‘ in Gondwana Research by Jain et al., 2019. Hot off the press, the first author of this study himself, Charitra Jain, Post Doctoral Research Associate in the Department of Earth Sciences at Durham University, shares the scoop in our News & Views!
Why do we care?
Uniquely positioned within the habitable zone [1], Earth is the sole planet within our solar system that sustains life. Understanding the factors that make a planet habitable [2,3] are becoming increasingly relevant with the rapidly expanding catalogue of extrasolar planets over the last decade [4,5]. The operation of plate tectonics and the formation and stability of continental landmasses have played a crucial role in the atmospheric evolution and development of life on Earth [6]. Plate tectonics is a theory where the outer surface of the Earth (lithosphere) is fragmented into a number of mobile plates that drift at a speed of few centimetres per year relative to each other, atop a convecting mantle. Mountains, volcanoes, and earthquakes are found at the boundaries of these plates. Continents cover about a third of the planet’s surface area and are made of thin crust overlying a thick and undeformable cratonic lithosphere [7,8]. Even after having assembled a reasonable model for its origins and internal workings, many fundamental questions pertaining to Earth sciences remain unresolved, for example:
- How did the first continents form and what accounts for their stability over billions of years? Was their growth an episodic process [9] or a continuous process with a significant drop in crustal growth rate around 3 Ga (billion years ago) [10]? How much of the continental crust has recycled into the mantle [11]?
- What was the global geodynamic regime exhibited by early Earth? When and how did the subduction-driven plate tectonics commence [12]? Did the presence of continents play a role in a regime transition from vertical tectonics to horizontal tectonics around 3Ga [13]?
Owing to the increasing paucity of natural observational data as we go back further in time [14], numerical modelling constrained by experimental and field data has thus become indispensable to uncover the secrets of Earth’s evolutionary history. Our recent study [15] is a step in the right direction where we have developed a new two-stage melting algorithm to create oceanic (basaltic/mafic) and continental (felsic) crust in self-consistent global mantle convection models for the first time.
What’s new in these models?
Generally, two stages of mantle differentiation are inferred to generate continental crust as shown in the schematic in Fig. 1A. First, basaltic magma is extracted from the mantle. Second, it is buried and partially melts to form felsic continental crust. During the much hotter Archean conditions [16,17], majority of continental crust was made of Tonalite-Trondhjemite-Granodiorite (TTG) [18,19] rocks. Experimental data suggests that TTGs are formed when hydrated basalt melts at garnet-amphibolite, granulite or eclogite facies conditions [20,21] and specific P-T conditions [22] have been employed as a criterion for generating TTGs by the authors in their models.
Interested in the long-term planetary evolution, we parametrised the processes of melt generation and melt extraction. If the melt is generated within the top 300 km of the mantle (Fig.1B1), it is instantaneously removed from the depth (Fig.1B2) [23,24] and transported both to the bottom of the crust (plutonism/intrusion) and to the top of the model domain (volcanism/eruption) (Fig. 1B3). The intruded melt stays molten while a temperature adjustment to account for adiabatic decompression is applied, and tends to result in a warm, weak lithosphere. The erupted melt is rapidly solidified by setting its temperature to the surface temperature (300K), resulting in a strong and cold lithosphere [25]. The mass ratio of erupted to intruded melt is controlled by a parameter called eruption efficiency, which is tested extensively in our simulations. Geological data suggests that the majority of mantle-derived melts intrude at a depth, corresponding to an eruption efficiency between 9% and 20% [26].
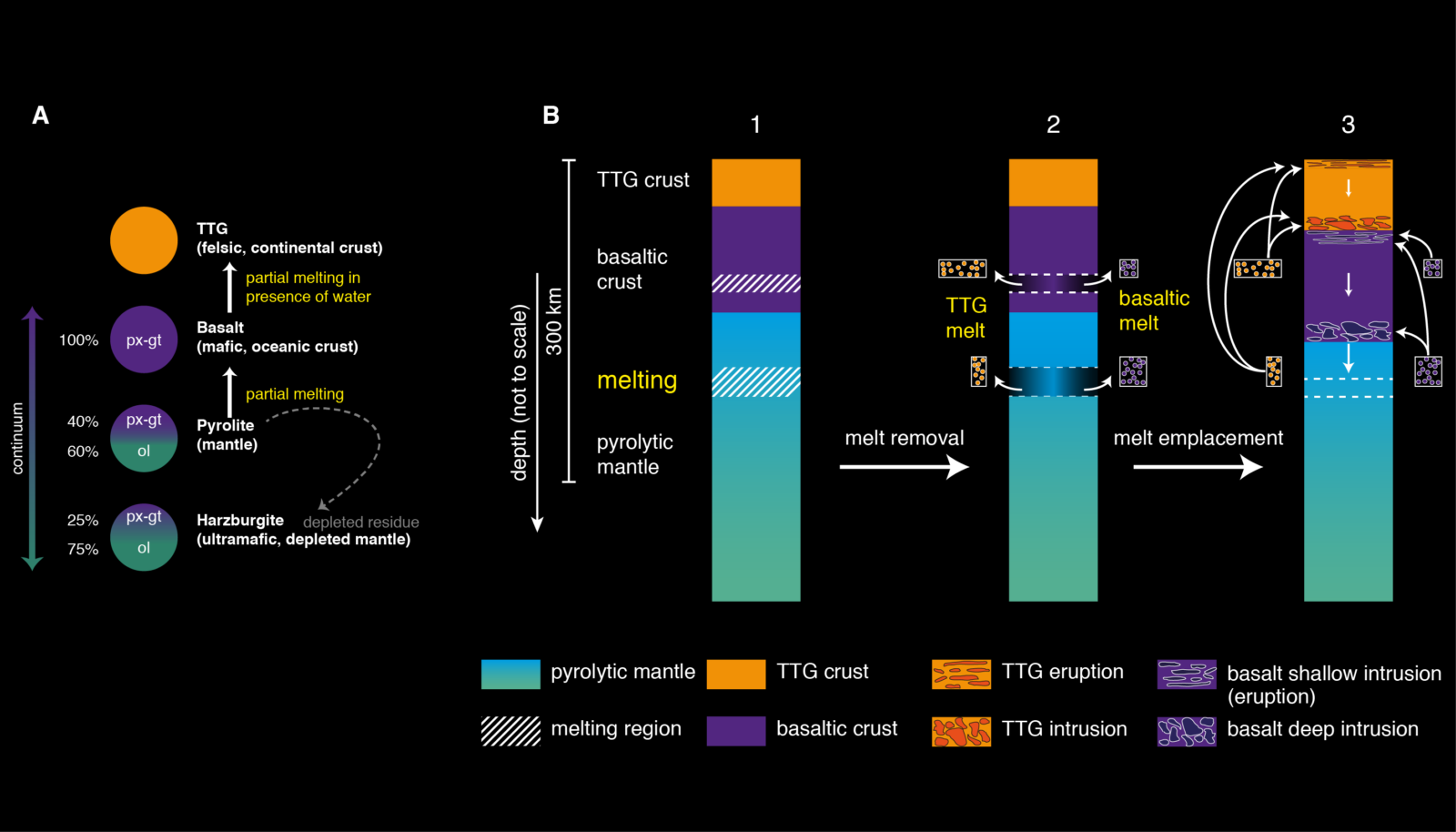
Figure 1: A, One-dimensional compositional variation with basalt-harzburgite continuum consisting of a mixture of olivine (ol) and pyroxene-garnet (px-gt) mineralogies in different proportions. Upon initialisa- tion, the whole mantle has a pyrolytic composition. B, Cartoon depicting a section of a mesh column (not to scale) in a stage: B1, where crustal production has already happened; B2, after melt removal but before compaction or opening gaps in lithosphere for magmatism; B3, with the eruption and intrusion of the melt with the white downgoing arrows representing compaction of tracers/markers.
How do these results stack up against data?
By varying initial core temperature, eruption efficiency, and limiting the mass of TTG that can be generated in our simulations to 10% and 50% of basalt mass, we present results from two sets of simulations. The crustal volumes have the same order of magnitude and the crustal composition follows similar trends as reported from geological and geochemical data [10,27,28]. Interestingly, we report two stages of TTG production without needing a significant change in convection regime: a period of continuous linear growth with time and intense recycling fuelled by strong plume activity and lasting for around 1 billion years, followed by a stage with reduced TTG growth and moderate recycling. The production of TTGs happen at the tip of deformation fronts driven by the lateral spreading of plumes (mantle upwellings) that rise to the surface (Fig. 2). These results indicate that the present-day slab- driven subduction was not required for the genesis of Archean TTGs [29,30] and early Earth exhibited a “plutonic squishy lid” or vertical-tectonics geodynamic regime [31–33].
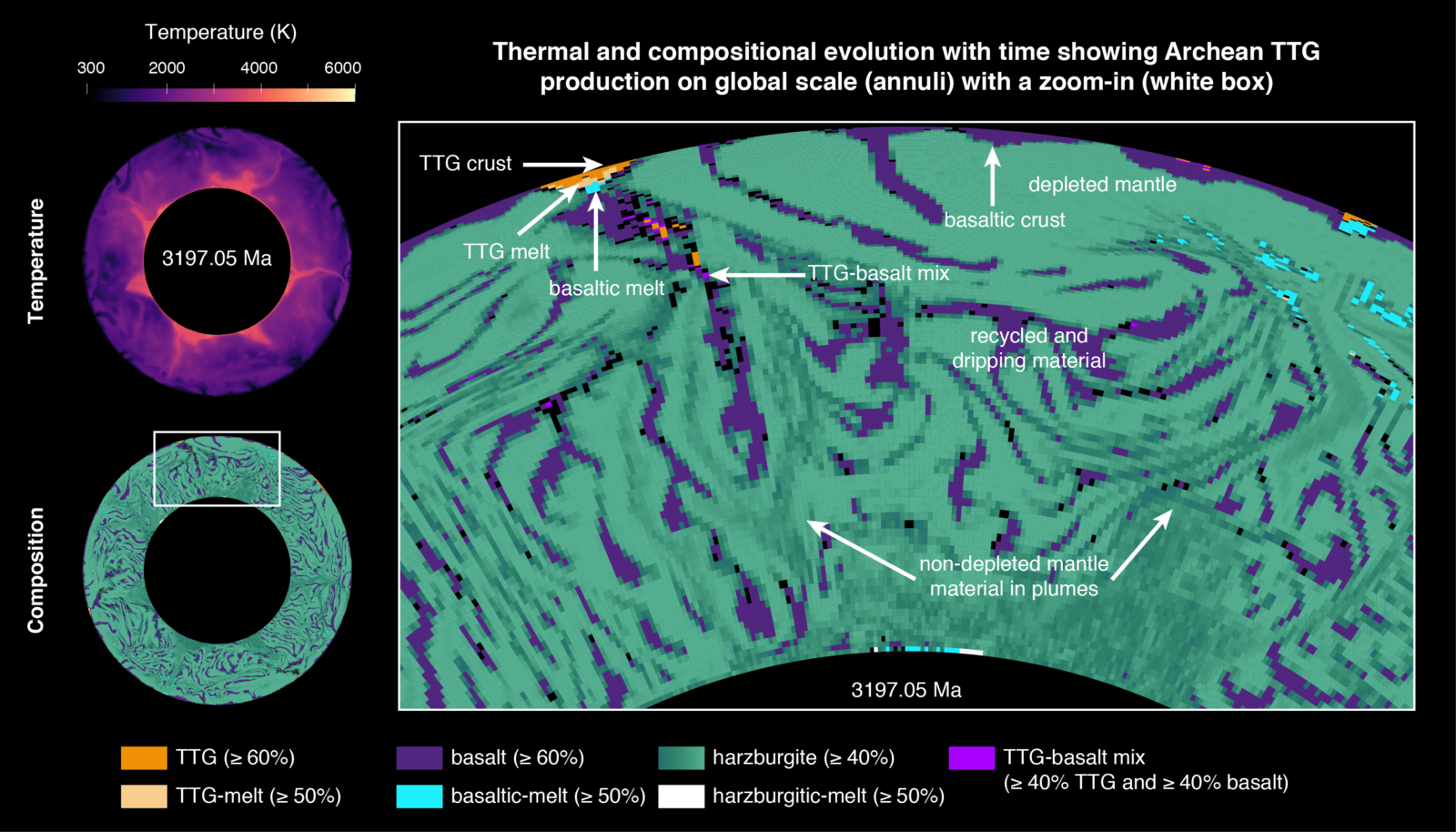
Figure 2: Thermal (top) and compositional (bottom and zoom-in) evolution with time for a simulation with initial core temperature of 6000 K, mantle potential temperature of 1900 K, eruption efficiency of 40%, and TTG mass limited to 10% of basalt mass. The lighter shades of teal in the composition field represent progressive mantle depletion (higher harzburgite content) with time.
What’s coming next?
These results are parameter-dependent to some extent and they would change in a 3D domain as that would limit the impact of plumes on lithosphere dynamics. Future models will aim for forming the viscous cratonic roots and incorporating the effects of water [34,35] and grain-size [36–38] on mantle rheology. Going forward, modelling coupled core-mantle-atmosphere systems will shed more light on the role of different tectonic modes towards planetary habitability [39,40] and help solve these contentious aspects.
References: [1] Huang, S.-S. The Problem of Life in the Universe and the Mode of Star Formation. Publications of the Astronomical Society of the Pacific 71, 421 (1959). [2] Kasting, J. F. & Catling, D. Evolution of a Habitable Planet. Annual Review of Astronomy and Astrophysics 41, 429–463 (2003). [3] Zahnle, K. et al. Emergence of a Habitable Planet. Space Science Reviews 129, 35–78 (2007). [4] Marcy, G. W. & Butler, R. P. Detection of extrasolar giant planets. Annual Review of Astronomy and Astrophysics 36, 57–97 (1998). [5] Gillon, M. et al. Seven temperate terrestrial planets around the nearby ultracool dwarf star TRAPPIST-1. Nature 542, 456–460 (2017). [6] Korenaga, J. Plate tectonics and planetary habitability: current status and future challenges. Annals of the New York Academy of Sciences 1260, 87–94 (2012). [7] Goodwin, A. M. Precambrian geology: the dynamic evolution of the continental crust (Academic Press, 1991). [8] Hoffmann, P. F. Precambrian geology and tectonic history of North America. Geology of North America—An Overview (Geological Society of America, 1989). [9] Condie, K. C. Supercontinents and superplume events: distinguishing signals in the geologic record. Physics of the Earth and Planetary Interiors 146, 319–332 (2004). [10] Dhuime, B., Hawkesworth, C. J., Delavault, H. & Cawood, P. A. Continental growth seen through the sedimentary record. Sedimentary Geology 357, 16–32 (2017). [11] Spencer, C. J., Roberts, N. M. W. & Santosh, M. Growth, destruction, and preservation of Earth’s continental crust. Earth Science Reviews 172, 87–106 (2017). [12] Korenaga, J. Initiation and Evolution of Plate Tectonics on Earth: Theories and Observations. Annual Review of Earth and Planetary Sciences 41, 117–151 (2013). [13] van Hunen, J., van Keken, P. E., Hynes, A. & Davies, G. F. Tectonics of early Earth: Some geodynamic considerations. In Special Paper 440: When Did Plate Tectonics Begin on Planet Earth?, 157–171 (Geological Society of America, 2008). [14] Gerya, T. Precambrian geodynamics: Concepts and models. Gondwana Research 25, 442–463 (2014). [15] Jain, C., Rozel, A. B., Tackley, P. J., Sanan, P. & Gerya, T. V. Growing primordial continental crust self-consistently in global mantle convection models. Gondwana Research 73, 96–122 (2019). [16] Labrosse, S. & Jaupart, C. Thermal evolution of the Earth: Secular changes and fluctuations of plate characteristics. Earth and Planetary Science Letters 260, 465–481 (2007). [17] Herzberg, C. & Gazel, E. Petrological evidence for secular cooling in mantle plumes. Nature 458, 619–622 (2009). [18] Jahn, B.-M., Glikson, A. Y., Peucat, J. J. & Hickman, A. H. REE geochemistry and isotopic data of Archean silicic volcanics and granitoids from the Pilbara Block, Western Australia: implications for the early crustal evolution. GEOCHIMICA ET COSMOCHIMICA ACTA 45, 1633–1652 (1981). [19] Drummond, M. S. & Defant, M. J. A model for Trondhjemite-Tonalite-Dacite Genesis and crustal growth via slab melting: Archean to modern comparisons. Journal of Geophysical Research 95, 21503–21521 (1990). [20] Barker, F. & Arth, J. G. Generation of trondhjemitic-tonalitic liquids and Archean bimodal trondhjemite-basalt suites. Geology 4, 596 (1976). [21] Martin, H. Effect of steeper Archean geothermal gradient on geochemistry of subduction-zone magmas. Geology 14, 753 (1986). [22] Moyen, J.-F. The composite Archaean grey gneisses: Petrological significance, and evidence for a non-unique tectonic setting for Archaean crustal growth. LITHOS 123, 21–36 (2011). [23] Christensen, U. R. & Hofmann, A. W. Segregation of subducted oceanic crust in the convecting mantle. Journal of Geophysical Research 99, 19867–19884 (1994). [24] Xie, S. & Tackley, P. J. Evolution of helium and argon isotopes in a convecting mantle. Physics of the Earth and Planetary Interiors 146, 417–439 (2004). [25] Rozel, A. B., Golabek, G. J., Jain, C., Tackley, P. J. & Gerya, T. Continental crust formation on early Earth controlled by intrusive magmatism. Nature 545, 332–335 (2017). 4 [26] Crisp, J. A. Rates of Magma Emplacement and Volcanic Output. Journal of Volcanology and Geothermal Research 20, 177–211 (1984). [27] Armstrong, R. L. Radiogenic Isotopes: The Case for Crustal Recycling on a Near-Steady-State No-Continental-Growth Earth. Philosophical Transactions of the Royal Society of London Series A: Mathematical Physical and Engineering Sciences 301, 443–472 (1981). [28] Tang, M., Chen, K. & Rudnick, R. L. Archean upper crust transition from mafic to felsic marks the onset of plate tectonics. Science 351, 372–375 (2016). [29] Bédard, J. H. A catalytic delamination-driven model for coupled genesis of Archaean crust and sub-continental litho- spheric mantle. GEOCHIMICA ET COSMOCHIMICA ACTA 70, 1188–1214 (2006). [30] Johnson, T. E., Brown, M., Gardiner, N. J., Kirkland, C. L. & Smithies, R. H. Earth’s first stable continents did not form by subduction. Nature 543, 239–242 (2017). [31] Van Kranendonk, M. J., Collins, W. J., Hickman, A. & Pawley, M. J. Critical tests of vertical vs. horizontal tectonic models for the Archaean East Pilbara Granite–Greenstone Terrane, Pilbara Craton, Western Australia. Precambrian research 131, 173–211 (2004). [32] Fischer, R. & Gerya, T. Early Earth plume-lid tectonics: A high-resolution 3D numerical modelling approach. Journal of Geodynamics 100, 198–214 (2016). [33] Lourenco, D. L., Rozel, A. B., Gerya, T. & Tackley, P. J. Efficient cooling of rocky planets by intrusive magmatism.Nature Geoscience 215, 1–6 (2018). [34] Hirth, G. & Kohlstedt, D. L. Water in the oceanic upper mantle: implications for rheology, melt extraction and the evolution of the lithosphere. Earth and Planetary Science Letters 144, 93–108 (1996). [35] Mei, S. & Kohlstedt, D. L. Influence of water on plastic deformation of olivine aggregates: 1. Diffusion creep regime.Journal of Geophysical Research 105, 21457–21469 (2000). [36] Hall, C. E. & Parmentier, E. M. Influence of grain size evolution on convective instability. Geochemistry, Geophysics, Geosystems 4, 469 (2003). [37] King, S. Archean cratons and mantle dynamics. Earth and Planetary Science Letters 234, 1–14 (2005). [38] Rozel, A. Impact of grain size on the convection of terrestrial planets. Geochemistry, Geophysics, Geosystems 13 (2012). [39] Gillmann, C. & Tackley, P. Atmosphere/mantle coupling and feedbacks on Venus. Journal of Geophysical Research: Planets 119, 1189–1217 (2014). [40] Foley, B. J. & Driscoll, P. E. Whole planet coupling between climate, mantle, and core: Implications for rocky planet evolution. Geochemistry, Geophysics, Geosystems 17, 1885–1914 (2016).